Within the pig family, most live on land but hippopotamuses have partly
returned
to live in water.
Richard-Dawkins-Unweaving-the-Rainbow
Finally, termites are rather special in that they themselves live in massive colonies of mostly sterile worker insects which plunder the country side more effectively than almost any other kind of animal except ants - and they are successful for the same kind of reason.
Mastotermes colonies can contain up to a million individual worker termites.
The species is a voracious pest in Australia, devouring telegraph poles, the plastic lining of electric cables, wooden buildings and bridges, even billiard balls.
Being a colony of colonies of colonies seems to be a successful recipe for life.
I want to return to the genes' eye view and push the idea of universal symbiosis - 'living together' - to its ultimate conclusion. Margulis is rightly seen as a high priestess of symbiosis. As I said earlier, I would go even further, and regard all 'normal' nuclear genes as symbiotic in the same kind of way as mitochondrial genes. But where Margulis and Lovelock invoke the poetry of cooperation and amity as primary in the union, I want to do the opposite and regard it as a secondary consequence. At the genetic level all is selfish, but the selfish ends of genes are served by cooperation at many levels. As far as the genes themselves are concerned, the relationships among our 'own' genes are not, in principle, different from the relationship between our genes and mitochondrial genes, or our genes and those of other species. All genes are being selected for their capacity to flourish in the presence of the other genes - of whatever species - whose consequences surround them.
Collaboration within gene pools to make complex bodies is often called co-adaptation, as distinct from co-evolution. Co-adaptation usually refers to the mutual tailoring of different bits of the same kind of organism to other bits. For example, many flowers have both a bright colour to attract insects and dark lines that act as runway guides to lead insects towards the nectar. Colour, lines and nectaries assist each other. They are co- adapted to each other, the genes that make them being selected in each others presence. Co-evolution is normally used to mean mutual evolution in different species. The flowers and the insects that pollinate them evolve together - co-evolve. In this case the co-evolutionary relationship is mutually beneficial. The word co-evolution is also used for the ' hostile kind of evolving together - co-evolutionary 'arms races'.
High-speed running in predators co-evolves with high-speed running in their prey. Thick armour co-evolves with weapons and techniques for penetrating it.
Although I have just made a clear distinction between 'within species' co- adaptation and 'between species' co-evolution, we can now see that a certain amount of confusion is pardonable. If we take the view, as I have
in this chapter, that gene interactions are just gene interactions, at any level, co-adaptation turns out to be just a special case of co-evolution. As far as the genes themselves are concerned, 'within species' is not fundamentally different from 'between species'. The differences are practical. Within a species, genes meet their companions inside cells. Between species, their consequences in the outside world may meet the consequences of the other genes, out in the external world. Intermediate cases, such as intimate parasites and mitochondria, are revealing because they blur the distinction.
Sceptics of natural selection often worry along the following lines. Natural selection, they say, is a purely negative process. It weeds out the unfit. How can such a negative weeding-out play the positive role of building up complex adaptation? A large part of the answer lies in a combination of co-evolution and co-adaptation, two processes which, as we have just seen, are not so very far apart.
Co-evolution, like a human arms race, is a recipe for progressive build- up of improvements (I mean improvements in efficiency at what they do, of course; obviously, from a humane point of view, 'improvements' in armaments are just the reverse). If predators get better at their job, prey have to follow suit just to stay in the same place. And vice versa. The same goes for parasites and hosts. Escalation begets further escalation. This leads to real progressive improvement in equipment for survival, even if it does not lead to improvement in survival itself (because, after all, the other side in the arms race is improving too). So, co-evolution - arms races, the mutual evolution of genes in different gene pools - is one answer to the sceptic who thinks natural selection is a purely negative process.
The other answer is co-adaptation, the mutual evolution of genes in the same gene pool. In the cheetah gene pool, carnivorous teeth work best with carnivorous guts and carnivorous habits. Herbivorous teeth, guts and habits form an alternative complex in an antelope gene pool. At the gene level, as we have seen, selection puts together harmonious complexes, not by choosing whole complexes but by favouring each part of the complex within gene pools that are dominated by the other parts of the complex. In the shifting balance of gene pools, more than one stable solution to the same problem may exist. Once a gene pool starts to become dominated by one stable solution, further selection of selfish genes favours the ingredients of the same solution. The other solution could equally well have been favoured if the starting conditions had been different. In any case, the sceptic's worry about whether natural selection is purely a negative, subtracting process is disarmed. Natural selection is positive and constructive. It is no more negative than a sculptor subtracting marble from a block. It carves out of gene pools complexes of
mutually interacting, co-adapted genes: fundamentally selfish but pragmatically cooperating. The unit that the Darwinian sculptor carves is the gene pool of a species.
I have devoted space in the last couple of chapters to warning of bad poetry in science. But the balance of my book is the opposite. Science is poetic, ought to be poetic, has much to learn from poets and should press good poetic imagery and metaphor into its inspirational service. 'The selfish gene' is a metaphoric image, potentially a good one but capable of sadly misleading if the metaphor of personification is improperly grasped. If interpreted aright it may lead us into paths of deep understanding and fertile research. This chapter has used the metaphor of the personified gene to explain a sense in which 'selfish' genes are also 'cooperative'.
The key image to be floated in the next chapter is that of a species' genes as a detailed description of the collection of environments in which its ancestors lived - a genetic book of the dead.
10
THE GENETIC BOOK OF THE DEAD
Remember the wisdom out of the old days . . .
W. B. YEATS, The Wind Among the Reeds (1899)
The first essay I can remember writing at school was 'The Diary of a Penny'. You had to imagine yourself a coin and tell your story, of how you sat in a bank for a while until you were given out to a customer, how it felt to jangle around in his pocket with the other coins, how you were handed over to buy something, then how you were passed out as change to another customer and then . . . well, you probably wrote a similar essay yourself. It is helpful to think the same way for a gene travelling not from pocket to pocket but from body to body down the generations. And the first point that the analogy of the coin makes is that of course the personification of the gene is not to be taken literally, any more than we seven-year-olds really thought our coins could talk. Personification is sometimes a useful device, and for critics to accuse us of taking it literally is almost as stupid as taking it literally in the first place. Physicists are not literally charmed by their particles, and the critic who would so accuse them is a tiresome pedant.
The 'minting' event for a gene is the mutation that brought it into existence by altering a previous gene. Only one of many copies of the gene in the population is changed (by one mutation event, but an
identical mutation may change another copy of the gene in the gene pool at another time). The others continue to make copies of the original gene, which may now be said to be in competition with the mutant form. Making copies is of course what genes, unlike coins, are supremely good at, and our diary of a gene has to include the experiences not of the particular atoms that go to make up the DNA, but the DNA's experiences in the form of multiple copies in successive generations. As the last chapter showed, much of a gene's 'experience' of past generations consists of rubbing up against other genes of the species, and this is why they cooperate so amicably in the collective enterprise of building bodies.
Now let's ask the question whether all the genes of a species have the same ancestral 'experiences'. Mostly they do. Most buffalo genes can look back to a long line of buffalo bodies which enjoyed, or suffered, common buffalo experiences. The bodies in which these genes survived included male and female buffaloes, large and small, and so on. But there are subsets of genes with different experiences, for example the genes that determine sex. In mammals, Y chromosomes are found only in males and do not exchange genes with other chromosomes. So a gene sitting on a Y chromosome has had a limited experience of buffalo bodies: male ones only. Its experiences are largely typical of buffalo genes in general, but not entirely so. Unlike most buffalo genes it doesn't know what it is like to sit in a female buffalo. A gene that has always been on a Y chromosome since the origin of the mammals during the age of dinosaurs will have experienced male bodies of many different species, but never a female body of any kind. The case of X chromosomes is more complicated to work out. Male mammals have one X chromosome (inherited from the mother, plus one Y chromosome inherited from the father), while females have two X chromosomes (one from each parent). So each X chromosome gene has experienced both female and male bodies, but two-thirds of its experience has been in female bodies. In birds the situation is reversed. The female bird has uneven sex chromosomes (which we may as well call X and Y by analogy with mammals, although the official bird terminology is different), the male two of the same (XX). The genes on the other chromosomes have all had an equal experience of male and female bodies, but their experiences may be unequal in other respects. A gene will have spent more than its fair share of time in ancestral bodies that possess whatever qualities the gene encodes - long legs, thick horns, or whatever it may be, especially if it is a dominant gene. Almost as obviously, all genes are likely to have spent more of their ancestral time in successful than in unsuccessful bodies. There are plenty of unsuccessful bodies about and they contain their full complement of genes. But they tend not to have descendants (that is what being unsuccessful means) so, as a gene looks back through its biography of past bodies, it will observe that all of them were as a matter of fact successful (by definition), and perhaps most (but not
all) of them were equipped with what it normally takes to be successful. The difference here is that individuals who are not equipped to be successful sometimes reproduce despite their lack. And individuals who are superbly equipped to survive and reproduce under average conditions are sometimes struck by lightning.
If, like some deer, seals and monkeys, the species is one in which the males form dominance hierarchies and dominant males do most of the reproducing, it will follow that the genes of the species will have more experience of dominant male bodies than of subordinate ones. (Note that we are no longer using dominant in its technical genetic sense, whose opposite is recessive, but in its ordinary language sense, where the opposite is subordinate. ) In every- generation, most of the males are subordinate, but their genes still look back on a strong line of dominant male ancestors. In every generation, the majority are fathered by a dominant minority from the previous generation. In the same way, if, like pheasants, the species is one in which, we suppose, most of the insemination is done by beautiful (to the females) males, most genes, whether they are now in females, in ugly males or in beautiful males, can look back on a long line of beautiful male ancestors. Genes have more experience of successful bodies than of unsuccessful ones.
To the extent that the genes of a species have regular and recurrent experience of subordinate bodies, we can expect to witness conditional strategies for 'making the best of a bad job'. In those species where successful males pugnaciously defend large harems, we sometimes notice subordinate males employing alternative, 'sneaky' strategies for gaining fleeting access to females. Seals have some of the most harem- dominated societies in the animal kingdom. In some populations, more than 90 per cent of the copulations are achieved by fewer than 10 per cent of the males. The bachelor majority of males, while biding their time awaiting their moment to depose one of the harem-bossing bulls, are alert for opportunities to sneak copulations with temporarily unguarded females. But, for such an alternative male strategy to have been favoured by natural selection, there must be at least a significant trickle of genes that have sneaked down the generations via stolen copulations. In our 'diary of a gene' language, then, at least some genes record subordinate males in their ancestral experience.
Do not be misled by the word 'experience'. It is not just that the word must be taken metaphorically rather than literally. That, I hope, is obvious. Less obvious is that we get a much more telling metaphor if we think of the whole species' gene pool, rather than a single gene, as the entity that gains experience from, its ancestral past. This is another aspect of our doctrine of 'the selfish cooperator'. Let me try and spell out what it means to say of a species, or its gene pool, that it learns from its
experiences. The species changes over evolutionary time. In any one generation, of course, the species consists of the set of its individual members alive at that time. Obviously this set changes as new members are born and old members die. This change in itself does not deserve to be called benefiting from experience, but the statistical distribution of genes in the population may systematically move in some specified direction, and that is 'species experience'. If an ice age is creeping up, more and more individuals will be seen to have thick hairy coats. Those individuals that happen to be the hairiest in any one generation tend to contribute more than their fair share of offspring, and hence genes for hairiness, to the next generation. The set of genes in the whole population - and therefore the genes likely to be contained in a typical average individual - becomes shifted in the direction of more and more genes for hairiness. The same thing is going on for other kinds of genes. As the generations go by, the whole set of genes of a species - the gene pool - is carved and whittled, kneaded and shaped, so that it becomes good at making successful individuals. It is in this sense that I say that the species is learning from its experience in the art of building good individual bodies, and it stores its experiences in coded form in the set of genes in the gene pool. Geological time is the timescale over which species become experienced. The information that the experience packs away is information about ancestral environments and how to survive them.
A species is an averaging computer. It builds up, over the generations, a statistical description of the worlds in which the ancestors of today's species members lived and reproduced. That description is written in the language of DNA. It lies not in the DNA of any one individual but collectively in the DNA - the selfish cooperators - of the whole breeding population. Perhaps 'read-out' captures it better than 'description'. If you find an animal's body, a new species previously unknown to science, a knowledgeable zoologist allowed to examine and dissect its every detail should be able to 'read' its body and tell you what kind of environment its ancestors inhabited: desert, rainforest, arctic tundra, temperate woodland or coral reef. The zoologist should also be able to tell you, by reading its teeth and its guts, what it fed on. Flat, millstone teeth and long intestines with complicated blind alleys indicate that it was a herbivore; sharp, shearing teeth and short, uncomplicated guts indicate a carnivore. The animal's feet, its eyes and other sense organs spell out the way it moved and how it found its food. Its stripes or flashes, its horns, antlers or crests, provide a read-out, for the knowledgeable, of its social and sex life.
But zoological science has a long way to go. Present day zoology can
'read' the body of a newly discovered species only to the extent of a rough, qualitative verdict about its probable habitat and way of life. The zoology
of the future will put into the computer many more measurements of the anatomy and chemistry of the animal being 'read'. More importantly, we shall not take the measurements separately. We shall perfect mathematical techniques of combining information from teeth, guts, stomach chemistry, social coloration and weapons, blood, bones,
muscles and ligaments. We shall incorporate methods of analysing the interactions of these measurements with one another. The computer, combining everything that is known about the body of the strange animal, will construct a detailed, quantitative model of the world, or worlds, in which the animal's ancestors survived. This, it seems to me, is tantamount to saying that the animal, any animal, is a model or description of its own world, or more precisely the worlds in which its ancestors' genes were naturally selected.
In a few cases, an animal's body is a description of the world in the literal sense of a pictorial representation. A stick insect lives in a world of twigs, and its body is a representational sculpture of a twig, leaf scars, buds and all. A fawn's pelage is a painting of the dappled pattern of sunlight filtered through trees on to the woodland floor. A peppered moth is a model of lichen on the tree bark. But just as art doesn't have to be literalist and representational, animals can be said to render their world in other ways: impressionistic, say, or symbolic. An artist seeking a dramatic impression of air speed could hardly do better than the shape of a swift. Perhaps this is because we have an intuitive understanding of streamlining; perhaps it is because we have grown used to the swept- back beauty of modem jet planes; perhaps it is because we have picked up some knowledge of the physics of turbulence and Reynolds Numbers, in which case we could say that the shape of the swift embodies coded facts about the viscosity of the air in which its ancestors flew. Whichever is the case, we see a swift as fitting the world of high-speed airflow as a hand fits a glove, an impression enhanced when we contrast it with the floundering clumsiness of a swift stranded on the ground and unable to take off.
A mole is not literally the shape of an underground tunnel. Perhaps it is a kind of negative image of a tunnel, shaped to squeeze through it. Its hands are not literally like soil, but they resemble spades which, through experience or intuition, we can see as the functional complement of soil: spades powered by heavy muscles to work against soil. There are even more striking cases where an animal, or a part of an animal, does not literally resemble its world but fits some part of it, glove-fashion. The coiled abdomen of a hermit crab is a coded representation of the mollusc shells in which its ancestors' genes lived. Or, we could say that the hermit crab's genes contain a coded prediction about an aspect of the world in which the crab will find itself. Because modern snails and
whelks are on average the same as ancient snails and whelks, hermit crabs still fit them and survive - the prediction is fulfilled.
Species of tiny mite are specialized to ride at a precise location on the inside of the pincer-like mandibles of a particular caste of army ant workers. Another species of mite is specialized to ride on the first joint of one antenna of an army ant. Each of these mites is shaped to fit its precise habitat, as a key fits a lock (Professor C. W. Rettenmeyer informs me - to my regret - that there are not mites shaped for left antennae and other mites shaped for right antennae). Just as a key embodies (complementary or negative) information about its own lock (information without which the door cannot be opened), so the mite embodies information about its world, in this case the shape of the insect joint where it lodges. (Parasites are often very specialized keys which fit their hosts' locks in much more detail than predators, presumably because it is unusual for a predator to attack only one species of host. The distinguished biologist Miriam Rothschild has a fund of delightful examples including a 'worm which lives exclusively under the eyelids of the hippopotamus and feeds upon its tears'. )
Sometimes the fit of animal to world is intuitively clear, either to common sense or to the trained eye of the engineer. Anybody can work out why webbed feet are so common among animals that frequently enter the water - ducks, platypuses, frogs, otters and others. If you are in any doubt, put on a pair of rubber frog feet and experience the sense of immediate release when you swim. You might even wish you had been born with frog feet, until you get out of the water and try to walk in your rubber ones. My friend Richard Leakey, paleoanthropologist, conservationist and African hero, lost both his legs in a light aircraft crash. Now he has two pairs of artificial legs: one pair with shoes, extra large for stability and permanently laced for walking, and another pair with flippers for swimming. Feet that are good for one way of life are bad for another. It is hard to design an animal that can do two such different things well.
Anybody can see why otters, seals and other air-breathing animals that live in water often have nostrils that can be closed at will. Again, human swimmers often resort to artifice, in this case a sprung nose-clip like a clothes peg. Anybody watching an ant-eater feeding through a hole in a nest of ants or termites can quickly see why they have been furnished with a long thin snout and sticky tongue. This is true not just of the specialized ant-eaters of South America, but of the unrelated pangolins and aardvark of Africa, and the even less closely related numbat and very distantly related spiny ant-eaters of Australasia. It is less obvious why all mammals that eat ants or termites have a low metabolic rate - a low body
temperature compared with most mammals, and a correspondingly low rate of biochemical turnover.
Our zoologists of the future, in order to reconstruct ancestral worlds and genetic descriptions of them, will need to replace intuitive common sense with systematic research. Here's how they might proceed. Begin by
listing a set of animals which are not particularly closely related to each other but which all share an important aspect of their life. Water- dwelling mammals would be a good test case. On more than a dozen separate occasions, land-dwelling mammals have returned to make their living, either wholly or partly, in water. We know they did so independently of each other because their closer cousins still live on land. The Pyrenean desman is a kind of aquatic mole, closely related to our familiar burrowing moles. Desmans and moles are members of the order Insectivora. Other members of the Insectivora who have independently evolved to live in fresh water include water shrews, one aquatic species of the exclusively Madagascan group of tenrecs, and three species of related otter shrews. That's four separate returnings to water among the Insectivora alone. All four are closer cousins to relatives living on dry
land than they are to the other freshwater species in the list. We have to count the three otter shrews as only a single returning to water because they are related to each other and presumably are all descended from a recent aquatic ancestor.
The surviving whales probably represent at most two separate returnings to water: the toothed whales (including dolphins) and the baleen whales. The surviving dugongs and manatees are close cousins of each other, and certainly their common ancestor also lived in the sea: they too, then, represent only a single returning to the sea.
Within the pig family, most live on land but hippopotamuses have partly returned to live in water. Beavers and true otters are other animals whose ancestors returned to the water. They can be directly compared with cousins who have stayed on land, say prairie dogs in the case of beavers, and badgers in the case of otters. Mink are members of the same genus as weasels and stoats (that puts them as close to each other as horses, zebras and donkeys are to each other) but they are semi-aquatic and have partially webbed feet. There is a South American water marsupial, the yapok, which can be directly compared with its land-dwelling cousins among the opossums. Among the egg-laying mammals of Australasia, the duck-billed platypuses live largely in water, the spiny ant-eaters on land. We can make a respectable list of matched pairs: each independently evolved aquatic group opposite the closest cousin we can find that has stayed on the land.
Given the list of matched pairs, we can immediately notice some obvious things. Most of the water-dwellers have at least partially webbed feet;
some have a tail that is modified into the shape of a paddle. These are obvious in the same kind of way as the long sticky tongue shared by ant- eaters. But, like the low metabolic rate shared by ant-eaters, there are probably less obvious characteristics shared by aquatic mammals as distinct from their terrestrial cousins. How shall we discover them? By a systematic statistical analysis; perhaps something like this.
Looking down our table of matched pairs, we make a large set of measurements, the same measurements for all the animals. We measure anything we can think of, with no prior expectations: pelvis width, eye radius, gut length, dozens more, all perhaps scaled as a ratio of total body size. Now throw all the measurements into the computer and invite it to work out which measurements need to be given high weighting in order to discriminate aquatic animals from their terrestrial cousins. We could calculate a number, the 'discrimination number', by summing up contributions from all the measurements, each one having been multiplied by a weighting factor. The computer adjusts the weighting given to each measurement, in order to maximize the difference, in the final sum, between aquatic mammals and their terrestrial opposite numbers. The foot webbing index will presumably emerge from the analysis with a strong weighting. The computer will discover that it pays - if you are trying to maximize the difference between aquatic and terrestrial animals - to multiply the webbing index by a high number before adding it to the discrimination number. Other measurements - things that mammals share without regard to the wetness of their world - will need to be multiplied by zero in order to eliminate their irrelevant and confusing contribution to the weighted sum.
At the end of the analysis we look down the weightings of all our measurements. Those that emerge with high weightings, like the foot webbing index, are the ones that have something to do with the wateriness of the way of life. Webbiness is obvious. What we hope is that the analysis will uncover other important discriminators that are not so obvious. Biochemical measures, for instance. When we have found them, we can then scratch our heads and wonder what connection they have with living in water or on land. This may suggest hypotheses for further research. Even if it doesn't, any measurement that gives us a statistically significant difference between animals that have adopted some way of life and their cousins that have not is very likely to be telling us something important about that way of life.
We can do the same thing with genes. Without any prior hypotheses about what the genes are doing, we make a systematic search for genetic resemblances between unrelated aquatic animals, which are not shared by their terrestrial close cousins. If we find any strong and statistically significant effects, even if we don't understand what those genes are
doing, I would say that what we are looking at might be regarded as a genetic description of watery worlds. Natural selection, to repeat, works as an averaging computer, doing the equivalent of a calculation that is not unlike the calculations we have just programmed our manmade computer to perform. Often a species embraces various ways of life, which may be radically different from each other. A caterpillar, and the butterfly it becomes, are members of the same species, yet our zoologist's reconstruction of their two ways of life would be utterly different. Caterpillar and butterfly contain exactly the same set of genes, and the genes must describe both environments, but separately. Presumably many of them are turned on in the plant-chewing, growing, caterpillar phase, and a largely different set of genes are turned on in the adult, reproductive, nectar-eating phase.
Male and female of most species live in at least somewhat different ways. The differences are pushed to extremes in angler fish, where the male appends himself as a tiny parasitic protuberance on the female's massive body. In most species, including ourselves, both male and female contain most of the genes for being either male or female. The differences lie in which genes are turned on. We all have genes for making penises and genes for making uteruses, regardless of our sex. ('Sex' is correct, by the way, not 'gender'. Gender is a grammatical technical term, applied to words not creatures. In German, a girl's gender is neuter but her sex female. Amerindian languages typically have two genders, animate and inanimate. The association of gender with sex in some groups of languages is incidental. It is quite a good joke that the politically inspired euphemism - saying gender when you mean sex - is consequently a piece of Western Imperialism. ) Our future zoologist reading the body of either a male or a female would get an incomplete picture of the ancestral worlds of the species. On the other hand, the genes of any member of the species would more nearly suffice to reconstruct a complete picture of the range of ways of life that the species has experienced.
Parasitic cuckoos are an oddity, and a fascinating one from the point of view of the Genetic Book of the Dead. As is well known, they are reared by foster parents of a species not their own. They never rear their own young. Not all are reared by the same foster species. In Britain, some are reared by meadow pipits, some by reed warblers, fewer by robins, some by a variety of other species, but the largest number are reared by dunnocks (hedge sparrows). As it happens, our foremost specialist on dunnocks and the author of Dunnock Behaviour and Social Evolution (1992) is also today's leading investigator of cuckoo biology, Nicholas Davies of Cambridge University. I shall base my account on the work of Davies and his colleague Michael Brooke because it lends itself especially well to being cast in the language of species 'experience' of ancestral
worlds. Unless otherwise stated I shall refer to the common cuckoo, Cuculus canorzis, in Britain.
Although they make mistakes on 10 per cent of occasions, a female cuckoo normally lays in the same kind of nest as her mother, her maternal grandmother, her maternal maternal great grandmother, and
so on. Presumably young females learn the characteristics of their foster nest and seek it out when their own time comes to lay. So, as far as females are concerned, there are dunnock cuckoos, reed warbler cuckoos, meadow pipit cuckoos, and so on, and they share this attribute with
their female-line relatives. But these are not separate species, not even separate races in the normal sense of the word. They are called 'gentes' (singular 'gens'). The reason a gens is not a true race or species is that male cuckoos don't belong to a gens. Since males don't lay eggs, they never have to choose a foster nest. And when a male cuckoo comes to mate, he just mates with a female cuckoo irrespective of her gens, and regardless of the foster species that reared either of them. It would follow from this that there is gene flow between the gentes. Males carry genes from one female gens to another. A female's mother, maternal grandmother and maternal maternal great grandmother will all belong to the same gens. But her paternal grandmother, both her paternal great grandmothers, and all her female ancestors to whom she is linked via
any male ancestor, could belong to any gens. From the point of view of gene 'experience', the consequence is very interesting. Recall that, in birds, it is the female sex that has the unequal sex chromosomes, X and Y, while male birds have two X chromosomes. Think what this means for the ancestral experience of genes on a Y chromosome. Since it passes unswervingly down the female line, never straying into the paths of male experience, a Y chromosome stays strictly within one gens. It is a dunnock cuckoo Y chromosome or a meadow pipit cuckoo Y chromosome. Its foster parent 'experience' is the same from generation to generation.
In this respect it differs from all other genes in the cuckoo, for they have all done time in male bodies and have hence shuffled freely around the female gentes, experiencing them all in proportion to their frequency.
In our language of genes as 'descriptions' of ancestral environments, most cuckoo genes will be in a position to describe those features that are shared by the complete range of foster nests that the species has parasitized. Y chromosome genes, uniquely, will describe only one type of foster nest, one species of foster parent. This means that Y chromosome genes, in a way that is not possible for other cuckoo genes, will be in a position to evolve specialized tricks for surviving in their own particular foster species' nest. What sort of tricks? Well, cuckoo eggs show at least some tendency to mimic the eggs of their foster species. Cuckoo eggs laid in meadow pipit nests are like large meadow pipit eggs. Cuckoo eggs laid in reed warbler nests are like large reed warbler eggs. Cuckoo eggs laid in
pied wagtail nests resemble pied wagtail eggs. Presumably this benefits the cuckoo eggs, which might otherwise be rejected by the foster parents. But think what it must mean from the genes' point of view.
If the genes for egg colour were on any chromosomes but the Y chromosome, they would be carried via males into the bodies of females belonging to the full assortment of gentes. This means they would be carried into the full range of host nests and there would be no consistent natural selection pressure to mimic one egg type more than another. It would be difficult for their eggs, in these circumstances, to mimic any but the most generalized features of all host eggs. Although there is no direct evidence, it is therefore reasonable to guess that the specific egg mimicry genes sit on the cuckoo Y chromosome. Females will then carry them, generation after generation, into nests of the same host. Their ancestral 'experience' will all be with the discriminating eyes of the same host, and those eyes will exert the selection pressure that steers their colour and spot pattern towards mimicry of host eggs.
There is a conspicuous exception. Cuckoo eggs laid in dunnock nests do not resemble dunnock eggs. They are no more variable among
themselves than eggs laid in reed warbler or meadow pipit nests; their colour is distinctive of the dunnock gens of cuckoos, and they don't
much resemble the eggs of any other gens, but they don't resemble dunnock eggs either. Why is this? It might be-thought that dunnock eggs, being a uniform pale blue, are harder to mimic than meadow pipit or
reed warbler eggs. Perhaps cuckoos just lack the physiological equipment to make plain blue eggs? I'm always suspicious of such last-resort theories, and in this case there is evidence against it. In Finland there is a gens of cuckoo that parasitizes redstarts, which also have plain blue eggs. These cuckoos, which belong to the same species as our British ones, succeed beautifully in matching redstart eggs. This surely shows that the failure of British cuckoos to mimic dunnock eggs cannot be put down to inherent incapacity to produce the unspeckled blue colour. Davies and Brooke believe that the true explanation lies in the recency of the relationship between dunnocks and cuckoos. Cuckoos run arms races in evolutionary time with each host species and the gens we are looking at has only recently 'invaded' dunnocks. Consequently,
dunnocks haven't yet had time to evolve counter-weapons. And dunnock cuckoos either haven't had time to evolve eggs that mimic dunnocks, or they don't yet need to because dunnocks haven't evolved the habit of discriminating foreign eggs from their own. In the language of this chapter, neither the dunnock gene pool nor the cuckoo gene pool (or rather, the Y chromosome of the dunnock cuckoo gens) has had enough experience of the other to evolve counter-weapons. Perhaps dunnock cuckoos are still adapted to outwit a different foster species, the one that their female ancestor left when she laid the first egg in a dunnock nest.
Meadow pipits, reed warblers and pied wagtails, on this view, are enemies of their respective gentes of cuckoos. There has been plenty of time for a build-up of weaponry on both sides. The hosts have built up keen eyes for an impostor egg and the cuckoos possess correspondingly cunning disguises for their eggs. Robins are an intermediate case. Their cuckoos lay eggs which are slightly robin-like, but not very. Perhaps the arms race between robins and the robin gens of cuckoos is of intermediate antiquity. On this view, the Y chromosomes of robin cuckoos are somewhat experienced, but their description of recent (robin) ancestral environments is still sketchy and contaminated by earlier descriptions of other species, previously 'experienced'.
Davies and Brooke did experiments deliberately putting extra eggs, of various kinds, in nests belonging to different species of birds. They wanted to see which species would accept, or reject, strange eggs. Their hypothesis was that species that have been through an arms race with cuckoos would, as a consequence of their genetic 'experience', be most likely to reject foreign eggs. One way to test this was to look at species which are not even suitable as cuckoo hosts. Baby cuckoos need to eat insects or worms. Species that feed their young on seeds, or species that nest in holes that female cuckoos can't reach, have never been at risk. Davies and Brooke predicted that such birds would not worry if they experimentally introduced strange eggs into their nests. And so it proved. Species that are suitable for cuckoos, however, like chaffinches, song thrushes and blackbirds, showed a stronger tendency to reject the experimental eggs that Davies and Brooke, playing cuckoo, placed in their nests. Flycatchers are potentially vulnerable in that they feed their young on a cuckoo-friendly diet. But whereas spotted flycatchers have open and accessible nests, pied flycatchers nest in holes which female cuckoos are too large to penetrate. Sure enough, when the experimenters dumped foreign eggs in their nests, pied flycatchers, with their 'inexperienced' gene pools, accepted foreign eggs without protest; spotted flycatchers, by contrast, rejected them, suggesting that their gene pools were wise to the cuckoo menace from long ago.
Davies and Brooke did similar experiments with species that cuckoos actually do parasitize. Meadow pipits, reed warblers and pied wagtails usually rejected artificially added eggs. As befits the 'lack of ancestral experience' hypothesis, dunnocks did not; nor did wrens. Robins and sedge warblers were intermediate. At the other extreme, reed buntings, which are suitable for cuckoos but not much parasitized by them, showed total rejection of foreign eggs. No wonder cuckoos don't parasitize them. Davies and Brooke's interpretation would presumably be that reed buntings have come out the other side of a long ancestral arms race with cuckoos, which they eventually won. Dunnocks are near the beginning of
their arms race. Robins are slightly more advanced in theirs. Meadow pipits, reed warblers and pied wagtails are in the middle of theirs. When we say dunnocks have only just begun their arms race with cuckoos, 'only just' has to be interpreted with evolutionary timescales in mind. By human standards the association could still be quite old. The Oxford English Dictionary quotes a 1616 reference to the Heisugge (archaic word for hedge sparrow or dunnock) as 'a bird which hatcheth the Cuckooes egges'. Davies notes the following lines in King Lear I, iv, written a decade earlier:
For, you trow, nuncle,
The hedge-sparrow fed the cuckoo so long,
That its had it head bit off by it young.
And in the fourteenth century Chaucer wrote of the cuckoo's treatment of the dunnock in The Parliament of Fowls:
'Thou mortherere of the hey sage on the braunche That broughte the forth, thow rewthelees glotoun! '
Although dunnock, hedge sparrow and heysoge are all given as synonyms in the dictionary, I can't help wondering how far we should rely on medieval ornithology. Chaucer himself was usually a rather precise user of language, but nevertheless the name sparrow has at times been given to what today is technically called an LBB (little brown bird). This may have been Shakespeare's meaning in the following, from Henry IV Part I, V, i:
And, being fed by us, you used us so
As that ungentle gull the cuckoo's bird,
Useth the sparrow - did oppress our nest
Grew by our feeding to so great a bulk
That even our love durst not come near your sight For fear of swallowing;
Sparrow, on its own, would nowadays mean the house sparrow, Passer domesticus, which is never parasitized by cuckoos. Despite its alternative name hedge sparrow, the dunnock, Prunella modularis, is unrelated; it is a 'sparrow' only in the loose sense of being a little brown bird. But
anyway, even if we take Chaucer's evidence as showing that the arms race between cuckoos and dunnocks really does go back at least to the fourteenth century, Davies and Brooke cite theoretical calculations, taking into account the comparative rarity of cuckoos, suggesting that this is still sufficiently recent in evolutionary terms to account for the apparent naivety of dunnocks when faced with cuckoos.
Before we leave cuckoos, here's an interesting thought. There could be, simultaneously existing, more than one gens of, say, robin cuckoos, who have built up their egg mimicry. ' independently. Since there is no gene flow between them as far as Y chromosomes are concerned, there could be accurate egg mimics coexisting with less accurate egg mimics. All are capable of mating with the same males but they don't share the same Y chromosomes. The accurate mimics would be descended from a female who moved into parasitizing robins a long time ago. The less accurate ones would be descended from a different female who moved into robins, possibly from a different predecessor host species, more recently.
Ants, termites and other social insect species are odd in a different way. They have sterile workers, often divided into several 'castes' - soldiers, media (middle-sized) workers, minor (small) workers, and so on. Every worker, whatever its caste, contains the genes that could have turned it into any other caste. Different sets of genes are switched on under different rearing conditions. It is by regulating these rearing conditions that the colony engineers a useful balance of different castes. Often the differences among castes are dramatic.
In the Asian ant species Pheidologeton diversus, the large worker caste (specialized for bulldozing smooth paths for other colony members) is 500 times heavier than the small caste, who do all the normal duties of a worker ant. The same set of genes equips a larva to grow up into either a Brobdingnagian or a Lilliputian, depending upon which ones are switched on. Honeypot ants are immobile storage vats, abdomens pumped up with nectar to transparent yellow spheres, hanging from the ceiling of the nest. The normal duties of an ants' nest, defence, foraging and, in this case, filling up the living vats, are done by normal workers whose abdomens are not swollen. The normal workers have genes that equip them to be honeypots, and honeypots, as far as their genes are concerned, could equally well be normal workers. As in the case of male and female, the visible differences in bodily form depend upon which genes are switched on. In this case it is determined by environmental factors, perhaps diet. Once again, the zoologist of the future could read out from the genes, but not the body, of any one member of the species a complete picture of the disparate lives of the different castes.
The European snail Cepaea nemoralis comes in a number of colours and patterns. The background shell colour can be any of six distinct shades (in order of dominance, in the technical genetic sense): brown, dark pink, light pink, very pale pink, dark yellow, light yellow. Overlaying this, there may be any number of stripes from zero to five. Unlike the case of the social insects, it is not true that every individual snail is genetically equipped to assume any of the different forms. Nor are these differences among snails determined by different environments of upbringing. Striped snails have genes that determine their number of stripes, dark pink individuals have genes that make them dark pink. But all the kinds can mate with each other.
The reasons for the persistence of many different types of snail (polymorphism), as well as the detailed genetics of the polymorphism itself, have been exhaustively studied by the English zoologists A. J. Cain and the late P. M. Sheppard with their school. A major part of the evolutionary explanation is that the species ranges over different habitats - woodland, grassland, bare soil - and you need a different colour pattern to be camouflaged against birds in each place. Beechwood snails contain an admixture of genes from grassland because they interbreed at the margins. A chalk downland snail has some genes that previously survived in the bodies of woodland ancestors; and their legacy, depending on the other genes in the snail, may be stripes. Our zoologist of the future would need to look at the gene pool of the species as a whole to reconstruct the full range of its ancestral worlds.
Just as Cepaea snails range over different habitats in space, so the ancestors of any species have changed their way of life from time to time. House mice, Mus musculus, today live almost exclusively in or around human habitations, as unwanted beneficiaries of human agriculture. But by evolutionary standards their way of life is recent. They must have fed on something else before there was human agriculture. Doubtless that something was sufficiently similar for their genetic skills to be pressed into service when the agricultural bonanza came along. Mice and rats have been described as animal weeds (incidentally, a good piece of poetic imagery, genuinely illuminating). They are generalists, opportunists, carrying genes that helped their ancestors to survive through probably a considerable range of ways of life; and pre-agricultural genes are in them yet. Anybody attempting to 'read' their genes may find a confusing palimpsest of ancestral world descriptions.
From earlier still, the DNA of all mammals must describe aspects of very ancient environments as well as more recent ones. The DNA of a camel was once in the sea, but it hasn't been there for a good 300 million years. It has spent most of recent geological history in deserts, programming bodies to withstand dust and conserve water. Like sandbluffs carved into
fantastic shapes by the desert winds, like rocks shaped by ocean waves, camel DNA has been sculpted by survival in ancient deserts, and even more ancient seas, to yield modern camels. Camel DNA speaks - if only we could understand the language - of the changing worlds of camel ancestors. If only we could read the language, the DNA of tuna and starfish would have 'sea' written into the text. The DNA of moles and earthworms would spell 'underground'. Of course all the DNA would spell many other things as well.
I want to return to the genes' eye view and push the idea of universal symbiosis - 'living together' - to its ultimate conclusion. Margulis is rightly seen as a high priestess of symbiosis. As I said earlier, I would go even further, and regard all 'normal' nuclear genes as symbiotic in the same kind of way as mitochondrial genes. But where Margulis and Lovelock invoke the poetry of cooperation and amity as primary in the union, I want to do the opposite and regard it as a secondary consequence. At the genetic level all is selfish, but the selfish ends of genes are served by cooperation at many levels. As far as the genes themselves are concerned, the relationships among our 'own' genes are not, in principle, different from the relationship between our genes and mitochondrial genes, or our genes and those of other species. All genes are being selected for their capacity to flourish in the presence of the other genes - of whatever species - whose consequences surround them.
Collaboration within gene pools to make complex bodies is often called co-adaptation, as distinct from co-evolution. Co-adaptation usually refers to the mutual tailoring of different bits of the same kind of organism to other bits. For example, many flowers have both a bright colour to attract insects and dark lines that act as runway guides to lead insects towards the nectar. Colour, lines and nectaries assist each other. They are co- adapted to each other, the genes that make them being selected in each others presence. Co-evolution is normally used to mean mutual evolution in different species. The flowers and the insects that pollinate them evolve together - co-evolve. In this case the co-evolutionary relationship is mutually beneficial. The word co-evolution is also used for the ' hostile kind of evolving together - co-evolutionary 'arms races'.
High-speed running in predators co-evolves with high-speed running in their prey. Thick armour co-evolves with weapons and techniques for penetrating it.
Although I have just made a clear distinction between 'within species' co- adaptation and 'between species' co-evolution, we can now see that a certain amount of confusion is pardonable. If we take the view, as I have
in this chapter, that gene interactions are just gene interactions, at any level, co-adaptation turns out to be just a special case of co-evolution. As far as the genes themselves are concerned, 'within species' is not fundamentally different from 'between species'. The differences are practical. Within a species, genes meet their companions inside cells. Between species, their consequences in the outside world may meet the consequences of the other genes, out in the external world. Intermediate cases, such as intimate parasites and mitochondria, are revealing because they blur the distinction.
Sceptics of natural selection often worry along the following lines. Natural selection, they say, is a purely negative process. It weeds out the unfit. How can such a negative weeding-out play the positive role of building up complex adaptation? A large part of the answer lies in a combination of co-evolution and co-adaptation, two processes which, as we have just seen, are not so very far apart.
Co-evolution, like a human arms race, is a recipe for progressive build- up of improvements (I mean improvements in efficiency at what they do, of course; obviously, from a humane point of view, 'improvements' in armaments are just the reverse). If predators get better at their job, prey have to follow suit just to stay in the same place. And vice versa. The same goes for parasites and hosts. Escalation begets further escalation. This leads to real progressive improvement in equipment for survival, even if it does not lead to improvement in survival itself (because, after all, the other side in the arms race is improving too). So, co-evolution - arms races, the mutual evolution of genes in different gene pools - is one answer to the sceptic who thinks natural selection is a purely negative process.
The other answer is co-adaptation, the mutual evolution of genes in the same gene pool. In the cheetah gene pool, carnivorous teeth work best with carnivorous guts and carnivorous habits. Herbivorous teeth, guts and habits form an alternative complex in an antelope gene pool. At the gene level, as we have seen, selection puts together harmonious complexes, not by choosing whole complexes but by favouring each part of the complex within gene pools that are dominated by the other parts of the complex. In the shifting balance of gene pools, more than one stable solution to the same problem may exist. Once a gene pool starts to become dominated by one stable solution, further selection of selfish genes favours the ingredients of the same solution. The other solution could equally well have been favoured if the starting conditions had been different. In any case, the sceptic's worry about whether natural selection is purely a negative, subtracting process is disarmed. Natural selection is positive and constructive. It is no more negative than a sculptor subtracting marble from a block. It carves out of gene pools complexes of
mutually interacting, co-adapted genes: fundamentally selfish but pragmatically cooperating. The unit that the Darwinian sculptor carves is the gene pool of a species.
I have devoted space in the last couple of chapters to warning of bad poetry in science. But the balance of my book is the opposite. Science is poetic, ought to be poetic, has much to learn from poets and should press good poetic imagery and metaphor into its inspirational service. 'The selfish gene' is a metaphoric image, potentially a good one but capable of sadly misleading if the metaphor of personification is improperly grasped. If interpreted aright it may lead us into paths of deep understanding and fertile research. This chapter has used the metaphor of the personified gene to explain a sense in which 'selfish' genes are also 'cooperative'.
The key image to be floated in the next chapter is that of a species' genes as a detailed description of the collection of environments in which its ancestors lived - a genetic book of the dead.
10
THE GENETIC BOOK OF THE DEAD
Remember the wisdom out of the old days . . .
W. B. YEATS, The Wind Among the Reeds (1899)
The first essay I can remember writing at school was 'The Diary of a Penny'. You had to imagine yourself a coin and tell your story, of how you sat in a bank for a while until you were given out to a customer, how it felt to jangle around in his pocket with the other coins, how you were handed over to buy something, then how you were passed out as change to another customer and then . . . well, you probably wrote a similar essay yourself. It is helpful to think the same way for a gene travelling not from pocket to pocket but from body to body down the generations. And the first point that the analogy of the coin makes is that of course the personification of the gene is not to be taken literally, any more than we seven-year-olds really thought our coins could talk. Personification is sometimes a useful device, and for critics to accuse us of taking it literally is almost as stupid as taking it literally in the first place. Physicists are not literally charmed by their particles, and the critic who would so accuse them is a tiresome pedant.
The 'minting' event for a gene is the mutation that brought it into existence by altering a previous gene. Only one of many copies of the gene in the population is changed (by one mutation event, but an
identical mutation may change another copy of the gene in the gene pool at another time). The others continue to make copies of the original gene, which may now be said to be in competition with the mutant form. Making copies is of course what genes, unlike coins, are supremely good at, and our diary of a gene has to include the experiences not of the particular atoms that go to make up the DNA, but the DNA's experiences in the form of multiple copies in successive generations. As the last chapter showed, much of a gene's 'experience' of past generations consists of rubbing up against other genes of the species, and this is why they cooperate so amicably in the collective enterprise of building bodies.
Now let's ask the question whether all the genes of a species have the same ancestral 'experiences'. Mostly they do. Most buffalo genes can look back to a long line of buffalo bodies which enjoyed, or suffered, common buffalo experiences. The bodies in which these genes survived included male and female buffaloes, large and small, and so on. But there are subsets of genes with different experiences, for example the genes that determine sex. In mammals, Y chromosomes are found only in males and do not exchange genes with other chromosomes. So a gene sitting on a Y chromosome has had a limited experience of buffalo bodies: male ones only. Its experiences are largely typical of buffalo genes in general, but not entirely so. Unlike most buffalo genes it doesn't know what it is like to sit in a female buffalo. A gene that has always been on a Y chromosome since the origin of the mammals during the age of dinosaurs will have experienced male bodies of many different species, but never a female body of any kind. The case of X chromosomes is more complicated to work out. Male mammals have one X chromosome (inherited from the mother, plus one Y chromosome inherited from the father), while females have two X chromosomes (one from each parent). So each X chromosome gene has experienced both female and male bodies, but two-thirds of its experience has been in female bodies. In birds the situation is reversed. The female bird has uneven sex chromosomes (which we may as well call X and Y by analogy with mammals, although the official bird terminology is different), the male two of the same (XX). The genes on the other chromosomes have all had an equal experience of male and female bodies, but their experiences may be unequal in other respects. A gene will have spent more than its fair share of time in ancestral bodies that possess whatever qualities the gene encodes - long legs, thick horns, or whatever it may be, especially if it is a dominant gene. Almost as obviously, all genes are likely to have spent more of their ancestral time in successful than in unsuccessful bodies. There are plenty of unsuccessful bodies about and they contain their full complement of genes. But they tend not to have descendants (that is what being unsuccessful means) so, as a gene looks back through its biography of past bodies, it will observe that all of them were as a matter of fact successful (by definition), and perhaps most (but not
all) of them were equipped with what it normally takes to be successful. The difference here is that individuals who are not equipped to be successful sometimes reproduce despite their lack. And individuals who are superbly equipped to survive and reproduce under average conditions are sometimes struck by lightning.
If, like some deer, seals and monkeys, the species is one in which the males form dominance hierarchies and dominant males do most of the reproducing, it will follow that the genes of the species will have more experience of dominant male bodies than of subordinate ones. (Note that we are no longer using dominant in its technical genetic sense, whose opposite is recessive, but in its ordinary language sense, where the opposite is subordinate. ) In every- generation, most of the males are subordinate, but their genes still look back on a strong line of dominant male ancestors. In every generation, the majority are fathered by a dominant minority from the previous generation. In the same way, if, like pheasants, the species is one in which, we suppose, most of the insemination is done by beautiful (to the females) males, most genes, whether they are now in females, in ugly males or in beautiful males, can look back on a long line of beautiful male ancestors. Genes have more experience of successful bodies than of unsuccessful ones.
To the extent that the genes of a species have regular and recurrent experience of subordinate bodies, we can expect to witness conditional strategies for 'making the best of a bad job'. In those species where successful males pugnaciously defend large harems, we sometimes notice subordinate males employing alternative, 'sneaky' strategies for gaining fleeting access to females. Seals have some of the most harem- dominated societies in the animal kingdom. In some populations, more than 90 per cent of the copulations are achieved by fewer than 10 per cent of the males. The bachelor majority of males, while biding their time awaiting their moment to depose one of the harem-bossing bulls, are alert for opportunities to sneak copulations with temporarily unguarded females. But, for such an alternative male strategy to have been favoured by natural selection, there must be at least a significant trickle of genes that have sneaked down the generations via stolen copulations. In our 'diary of a gene' language, then, at least some genes record subordinate males in their ancestral experience.
Do not be misled by the word 'experience'. It is not just that the word must be taken metaphorically rather than literally. That, I hope, is obvious. Less obvious is that we get a much more telling metaphor if we think of the whole species' gene pool, rather than a single gene, as the entity that gains experience from, its ancestral past. This is another aspect of our doctrine of 'the selfish cooperator'. Let me try and spell out what it means to say of a species, or its gene pool, that it learns from its
experiences. The species changes over evolutionary time. In any one generation, of course, the species consists of the set of its individual members alive at that time. Obviously this set changes as new members are born and old members die. This change in itself does not deserve to be called benefiting from experience, but the statistical distribution of genes in the population may systematically move in some specified direction, and that is 'species experience'. If an ice age is creeping up, more and more individuals will be seen to have thick hairy coats. Those individuals that happen to be the hairiest in any one generation tend to contribute more than their fair share of offspring, and hence genes for hairiness, to the next generation. The set of genes in the whole population - and therefore the genes likely to be contained in a typical average individual - becomes shifted in the direction of more and more genes for hairiness. The same thing is going on for other kinds of genes. As the generations go by, the whole set of genes of a species - the gene pool - is carved and whittled, kneaded and shaped, so that it becomes good at making successful individuals. It is in this sense that I say that the species is learning from its experience in the art of building good individual bodies, and it stores its experiences in coded form in the set of genes in the gene pool. Geological time is the timescale over which species become experienced. The information that the experience packs away is information about ancestral environments and how to survive them.
A species is an averaging computer. It builds up, over the generations, a statistical description of the worlds in which the ancestors of today's species members lived and reproduced. That description is written in the language of DNA. It lies not in the DNA of any one individual but collectively in the DNA - the selfish cooperators - of the whole breeding population. Perhaps 'read-out' captures it better than 'description'. If you find an animal's body, a new species previously unknown to science, a knowledgeable zoologist allowed to examine and dissect its every detail should be able to 'read' its body and tell you what kind of environment its ancestors inhabited: desert, rainforest, arctic tundra, temperate woodland or coral reef. The zoologist should also be able to tell you, by reading its teeth and its guts, what it fed on. Flat, millstone teeth and long intestines with complicated blind alleys indicate that it was a herbivore; sharp, shearing teeth and short, uncomplicated guts indicate a carnivore. The animal's feet, its eyes and other sense organs spell out the way it moved and how it found its food. Its stripes or flashes, its horns, antlers or crests, provide a read-out, for the knowledgeable, of its social and sex life.
But zoological science has a long way to go. Present day zoology can
'read' the body of a newly discovered species only to the extent of a rough, qualitative verdict about its probable habitat and way of life. The zoology
of the future will put into the computer many more measurements of the anatomy and chemistry of the animal being 'read'. More importantly, we shall not take the measurements separately. We shall perfect mathematical techniques of combining information from teeth, guts, stomach chemistry, social coloration and weapons, blood, bones,
muscles and ligaments. We shall incorporate methods of analysing the interactions of these measurements with one another. The computer, combining everything that is known about the body of the strange animal, will construct a detailed, quantitative model of the world, or worlds, in which the animal's ancestors survived. This, it seems to me, is tantamount to saying that the animal, any animal, is a model or description of its own world, or more precisely the worlds in which its ancestors' genes were naturally selected.
In a few cases, an animal's body is a description of the world in the literal sense of a pictorial representation. A stick insect lives in a world of twigs, and its body is a representational sculpture of a twig, leaf scars, buds and all. A fawn's pelage is a painting of the dappled pattern of sunlight filtered through trees on to the woodland floor. A peppered moth is a model of lichen on the tree bark. But just as art doesn't have to be literalist and representational, animals can be said to render their world in other ways: impressionistic, say, or symbolic. An artist seeking a dramatic impression of air speed could hardly do better than the shape of a swift. Perhaps this is because we have an intuitive understanding of streamlining; perhaps it is because we have grown used to the swept- back beauty of modem jet planes; perhaps it is because we have picked up some knowledge of the physics of turbulence and Reynolds Numbers, in which case we could say that the shape of the swift embodies coded facts about the viscosity of the air in which its ancestors flew. Whichever is the case, we see a swift as fitting the world of high-speed airflow as a hand fits a glove, an impression enhanced when we contrast it with the floundering clumsiness of a swift stranded on the ground and unable to take off.
A mole is not literally the shape of an underground tunnel. Perhaps it is a kind of negative image of a tunnel, shaped to squeeze through it. Its hands are not literally like soil, but they resemble spades which, through experience or intuition, we can see as the functional complement of soil: spades powered by heavy muscles to work against soil. There are even more striking cases where an animal, or a part of an animal, does not literally resemble its world but fits some part of it, glove-fashion. The coiled abdomen of a hermit crab is a coded representation of the mollusc shells in which its ancestors' genes lived. Or, we could say that the hermit crab's genes contain a coded prediction about an aspect of the world in which the crab will find itself. Because modern snails and
whelks are on average the same as ancient snails and whelks, hermit crabs still fit them and survive - the prediction is fulfilled.
Species of tiny mite are specialized to ride at a precise location on the inside of the pincer-like mandibles of a particular caste of army ant workers. Another species of mite is specialized to ride on the first joint of one antenna of an army ant. Each of these mites is shaped to fit its precise habitat, as a key fits a lock (Professor C. W. Rettenmeyer informs me - to my regret - that there are not mites shaped for left antennae and other mites shaped for right antennae). Just as a key embodies (complementary or negative) information about its own lock (information without which the door cannot be opened), so the mite embodies information about its world, in this case the shape of the insect joint where it lodges. (Parasites are often very specialized keys which fit their hosts' locks in much more detail than predators, presumably because it is unusual for a predator to attack only one species of host. The distinguished biologist Miriam Rothschild has a fund of delightful examples including a 'worm which lives exclusively under the eyelids of the hippopotamus and feeds upon its tears'. )
Sometimes the fit of animal to world is intuitively clear, either to common sense or to the trained eye of the engineer. Anybody can work out why webbed feet are so common among animals that frequently enter the water - ducks, platypuses, frogs, otters and others. If you are in any doubt, put on a pair of rubber frog feet and experience the sense of immediate release when you swim. You might even wish you had been born with frog feet, until you get out of the water and try to walk in your rubber ones. My friend Richard Leakey, paleoanthropologist, conservationist and African hero, lost both his legs in a light aircraft crash. Now he has two pairs of artificial legs: one pair with shoes, extra large for stability and permanently laced for walking, and another pair with flippers for swimming. Feet that are good for one way of life are bad for another. It is hard to design an animal that can do two such different things well.
Anybody can see why otters, seals and other air-breathing animals that live in water often have nostrils that can be closed at will. Again, human swimmers often resort to artifice, in this case a sprung nose-clip like a clothes peg. Anybody watching an ant-eater feeding through a hole in a nest of ants or termites can quickly see why they have been furnished with a long thin snout and sticky tongue. This is true not just of the specialized ant-eaters of South America, but of the unrelated pangolins and aardvark of Africa, and the even less closely related numbat and very distantly related spiny ant-eaters of Australasia. It is less obvious why all mammals that eat ants or termites have a low metabolic rate - a low body
temperature compared with most mammals, and a correspondingly low rate of biochemical turnover.
Our zoologists of the future, in order to reconstruct ancestral worlds and genetic descriptions of them, will need to replace intuitive common sense with systematic research. Here's how they might proceed. Begin by
listing a set of animals which are not particularly closely related to each other but which all share an important aspect of their life. Water- dwelling mammals would be a good test case. On more than a dozen separate occasions, land-dwelling mammals have returned to make their living, either wholly or partly, in water. We know they did so independently of each other because their closer cousins still live on land. The Pyrenean desman is a kind of aquatic mole, closely related to our familiar burrowing moles. Desmans and moles are members of the order Insectivora. Other members of the Insectivora who have independently evolved to live in fresh water include water shrews, one aquatic species of the exclusively Madagascan group of tenrecs, and three species of related otter shrews. That's four separate returnings to water among the Insectivora alone. All four are closer cousins to relatives living on dry
land than they are to the other freshwater species in the list. We have to count the three otter shrews as only a single returning to water because they are related to each other and presumably are all descended from a recent aquatic ancestor.
The surviving whales probably represent at most two separate returnings to water: the toothed whales (including dolphins) and the baleen whales. The surviving dugongs and manatees are close cousins of each other, and certainly their common ancestor also lived in the sea: they too, then, represent only a single returning to the sea.
Within the pig family, most live on land but hippopotamuses have partly returned to live in water. Beavers and true otters are other animals whose ancestors returned to the water. They can be directly compared with cousins who have stayed on land, say prairie dogs in the case of beavers, and badgers in the case of otters. Mink are members of the same genus as weasels and stoats (that puts them as close to each other as horses, zebras and donkeys are to each other) but they are semi-aquatic and have partially webbed feet. There is a South American water marsupial, the yapok, which can be directly compared with its land-dwelling cousins among the opossums. Among the egg-laying mammals of Australasia, the duck-billed platypuses live largely in water, the spiny ant-eaters on land. We can make a respectable list of matched pairs: each independently evolved aquatic group opposite the closest cousin we can find that has stayed on the land.
Given the list of matched pairs, we can immediately notice some obvious things. Most of the water-dwellers have at least partially webbed feet;
some have a tail that is modified into the shape of a paddle. These are obvious in the same kind of way as the long sticky tongue shared by ant- eaters. But, like the low metabolic rate shared by ant-eaters, there are probably less obvious characteristics shared by aquatic mammals as distinct from their terrestrial cousins. How shall we discover them? By a systematic statistical analysis; perhaps something like this.
Looking down our table of matched pairs, we make a large set of measurements, the same measurements for all the animals. We measure anything we can think of, with no prior expectations: pelvis width, eye radius, gut length, dozens more, all perhaps scaled as a ratio of total body size. Now throw all the measurements into the computer and invite it to work out which measurements need to be given high weighting in order to discriminate aquatic animals from their terrestrial cousins. We could calculate a number, the 'discrimination number', by summing up contributions from all the measurements, each one having been multiplied by a weighting factor. The computer adjusts the weighting given to each measurement, in order to maximize the difference, in the final sum, between aquatic mammals and their terrestrial opposite numbers. The foot webbing index will presumably emerge from the analysis with a strong weighting. The computer will discover that it pays - if you are trying to maximize the difference between aquatic and terrestrial animals - to multiply the webbing index by a high number before adding it to the discrimination number. Other measurements - things that mammals share without regard to the wetness of their world - will need to be multiplied by zero in order to eliminate their irrelevant and confusing contribution to the weighted sum.
At the end of the analysis we look down the weightings of all our measurements. Those that emerge with high weightings, like the foot webbing index, are the ones that have something to do with the wateriness of the way of life. Webbiness is obvious. What we hope is that the analysis will uncover other important discriminators that are not so obvious. Biochemical measures, for instance. When we have found them, we can then scratch our heads and wonder what connection they have with living in water or on land. This may suggest hypotheses for further research. Even if it doesn't, any measurement that gives us a statistically significant difference between animals that have adopted some way of life and their cousins that have not is very likely to be telling us something important about that way of life.
We can do the same thing with genes. Without any prior hypotheses about what the genes are doing, we make a systematic search for genetic resemblances between unrelated aquatic animals, which are not shared by their terrestrial close cousins. If we find any strong and statistically significant effects, even if we don't understand what those genes are
doing, I would say that what we are looking at might be regarded as a genetic description of watery worlds. Natural selection, to repeat, works as an averaging computer, doing the equivalent of a calculation that is not unlike the calculations we have just programmed our manmade computer to perform. Often a species embraces various ways of life, which may be radically different from each other. A caterpillar, and the butterfly it becomes, are members of the same species, yet our zoologist's reconstruction of their two ways of life would be utterly different. Caterpillar and butterfly contain exactly the same set of genes, and the genes must describe both environments, but separately. Presumably many of them are turned on in the plant-chewing, growing, caterpillar phase, and a largely different set of genes are turned on in the adult, reproductive, nectar-eating phase.
Male and female of most species live in at least somewhat different ways. The differences are pushed to extremes in angler fish, where the male appends himself as a tiny parasitic protuberance on the female's massive body. In most species, including ourselves, both male and female contain most of the genes for being either male or female. The differences lie in which genes are turned on. We all have genes for making penises and genes for making uteruses, regardless of our sex. ('Sex' is correct, by the way, not 'gender'. Gender is a grammatical technical term, applied to words not creatures. In German, a girl's gender is neuter but her sex female. Amerindian languages typically have two genders, animate and inanimate. The association of gender with sex in some groups of languages is incidental. It is quite a good joke that the politically inspired euphemism - saying gender when you mean sex - is consequently a piece of Western Imperialism. ) Our future zoologist reading the body of either a male or a female would get an incomplete picture of the ancestral worlds of the species. On the other hand, the genes of any member of the species would more nearly suffice to reconstruct a complete picture of the range of ways of life that the species has experienced.
Parasitic cuckoos are an oddity, and a fascinating one from the point of view of the Genetic Book of the Dead. As is well known, they are reared by foster parents of a species not their own. They never rear their own young. Not all are reared by the same foster species. In Britain, some are reared by meadow pipits, some by reed warblers, fewer by robins, some by a variety of other species, but the largest number are reared by dunnocks (hedge sparrows). As it happens, our foremost specialist on dunnocks and the author of Dunnock Behaviour and Social Evolution (1992) is also today's leading investigator of cuckoo biology, Nicholas Davies of Cambridge University. I shall base my account on the work of Davies and his colleague Michael Brooke because it lends itself especially well to being cast in the language of species 'experience' of ancestral
worlds. Unless otherwise stated I shall refer to the common cuckoo, Cuculus canorzis, in Britain.
Although they make mistakes on 10 per cent of occasions, a female cuckoo normally lays in the same kind of nest as her mother, her maternal grandmother, her maternal maternal great grandmother, and
so on. Presumably young females learn the characteristics of their foster nest and seek it out when their own time comes to lay. So, as far as females are concerned, there are dunnock cuckoos, reed warbler cuckoos, meadow pipit cuckoos, and so on, and they share this attribute with
their female-line relatives. But these are not separate species, not even separate races in the normal sense of the word. They are called 'gentes' (singular 'gens'). The reason a gens is not a true race or species is that male cuckoos don't belong to a gens. Since males don't lay eggs, they never have to choose a foster nest. And when a male cuckoo comes to mate, he just mates with a female cuckoo irrespective of her gens, and regardless of the foster species that reared either of them. It would follow from this that there is gene flow between the gentes. Males carry genes from one female gens to another. A female's mother, maternal grandmother and maternal maternal great grandmother will all belong to the same gens. But her paternal grandmother, both her paternal great grandmothers, and all her female ancestors to whom she is linked via
any male ancestor, could belong to any gens. From the point of view of gene 'experience', the consequence is very interesting. Recall that, in birds, it is the female sex that has the unequal sex chromosomes, X and Y, while male birds have two X chromosomes. Think what this means for the ancestral experience of genes on a Y chromosome. Since it passes unswervingly down the female line, never straying into the paths of male experience, a Y chromosome stays strictly within one gens. It is a dunnock cuckoo Y chromosome or a meadow pipit cuckoo Y chromosome. Its foster parent 'experience' is the same from generation to generation.
In this respect it differs from all other genes in the cuckoo, for they have all done time in male bodies and have hence shuffled freely around the female gentes, experiencing them all in proportion to their frequency.
In our language of genes as 'descriptions' of ancestral environments, most cuckoo genes will be in a position to describe those features that are shared by the complete range of foster nests that the species has parasitized. Y chromosome genes, uniquely, will describe only one type of foster nest, one species of foster parent. This means that Y chromosome genes, in a way that is not possible for other cuckoo genes, will be in a position to evolve specialized tricks for surviving in their own particular foster species' nest. What sort of tricks? Well, cuckoo eggs show at least some tendency to mimic the eggs of their foster species. Cuckoo eggs laid in meadow pipit nests are like large meadow pipit eggs. Cuckoo eggs laid in reed warbler nests are like large reed warbler eggs. Cuckoo eggs laid in
pied wagtail nests resemble pied wagtail eggs. Presumably this benefits the cuckoo eggs, which might otherwise be rejected by the foster parents. But think what it must mean from the genes' point of view.
If the genes for egg colour were on any chromosomes but the Y chromosome, they would be carried via males into the bodies of females belonging to the full assortment of gentes. This means they would be carried into the full range of host nests and there would be no consistent natural selection pressure to mimic one egg type more than another. It would be difficult for their eggs, in these circumstances, to mimic any but the most generalized features of all host eggs. Although there is no direct evidence, it is therefore reasonable to guess that the specific egg mimicry genes sit on the cuckoo Y chromosome. Females will then carry them, generation after generation, into nests of the same host. Their ancestral 'experience' will all be with the discriminating eyes of the same host, and those eyes will exert the selection pressure that steers their colour and spot pattern towards mimicry of host eggs.
There is a conspicuous exception. Cuckoo eggs laid in dunnock nests do not resemble dunnock eggs. They are no more variable among
themselves than eggs laid in reed warbler or meadow pipit nests; their colour is distinctive of the dunnock gens of cuckoos, and they don't
much resemble the eggs of any other gens, but they don't resemble dunnock eggs either. Why is this? It might be-thought that dunnock eggs, being a uniform pale blue, are harder to mimic than meadow pipit or
reed warbler eggs. Perhaps cuckoos just lack the physiological equipment to make plain blue eggs? I'm always suspicious of such last-resort theories, and in this case there is evidence against it. In Finland there is a gens of cuckoo that parasitizes redstarts, which also have plain blue eggs. These cuckoos, which belong to the same species as our British ones, succeed beautifully in matching redstart eggs. This surely shows that the failure of British cuckoos to mimic dunnock eggs cannot be put down to inherent incapacity to produce the unspeckled blue colour. Davies and Brooke believe that the true explanation lies in the recency of the relationship between dunnocks and cuckoos. Cuckoos run arms races in evolutionary time with each host species and the gens we are looking at has only recently 'invaded' dunnocks. Consequently,
dunnocks haven't yet had time to evolve counter-weapons. And dunnock cuckoos either haven't had time to evolve eggs that mimic dunnocks, or they don't yet need to because dunnocks haven't evolved the habit of discriminating foreign eggs from their own. In the language of this chapter, neither the dunnock gene pool nor the cuckoo gene pool (or rather, the Y chromosome of the dunnock cuckoo gens) has had enough experience of the other to evolve counter-weapons. Perhaps dunnock cuckoos are still adapted to outwit a different foster species, the one that their female ancestor left when she laid the first egg in a dunnock nest.
Meadow pipits, reed warblers and pied wagtails, on this view, are enemies of their respective gentes of cuckoos. There has been plenty of time for a build-up of weaponry on both sides. The hosts have built up keen eyes for an impostor egg and the cuckoos possess correspondingly cunning disguises for their eggs. Robins are an intermediate case. Their cuckoos lay eggs which are slightly robin-like, but not very. Perhaps the arms race between robins and the robin gens of cuckoos is of intermediate antiquity. On this view, the Y chromosomes of robin cuckoos are somewhat experienced, but their description of recent (robin) ancestral environments is still sketchy and contaminated by earlier descriptions of other species, previously 'experienced'.
Davies and Brooke did experiments deliberately putting extra eggs, of various kinds, in nests belonging to different species of birds. They wanted to see which species would accept, or reject, strange eggs. Their hypothesis was that species that have been through an arms race with cuckoos would, as a consequence of their genetic 'experience', be most likely to reject foreign eggs. One way to test this was to look at species which are not even suitable as cuckoo hosts. Baby cuckoos need to eat insects or worms. Species that feed their young on seeds, or species that nest in holes that female cuckoos can't reach, have never been at risk. Davies and Brooke predicted that such birds would not worry if they experimentally introduced strange eggs into their nests. And so it proved. Species that are suitable for cuckoos, however, like chaffinches, song thrushes and blackbirds, showed a stronger tendency to reject the experimental eggs that Davies and Brooke, playing cuckoo, placed in their nests. Flycatchers are potentially vulnerable in that they feed their young on a cuckoo-friendly diet. But whereas spotted flycatchers have open and accessible nests, pied flycatchers nest in holes which female cuckoos are too large to penetrate. Sure enough, when the experimenters dumped foreign eggs in their nests, pied flycatchers, with their 'inexperienced' gene pools, accepted foreign eggs without protest; spotted flycatchers, by contrast, rejected them, suggesting that their gene pools were wise to the cuckoo menace from long ago.
Davies and Brooke did similar experiments with species that cuckoos actually do parasitize. Meadow pipits, reed warblers and pied wagtails usually rejected artificially added eggs. As befits the 'lack of ancestral experience' hypothesis, dunnocks did not; nor did wrens. Robins and sedge warblers were intermediate. At the other extreme, reed buntings, which are suitable for cuckoos but not much parasitized by them, showed total rejection of foreign eggs. No wonder cuckoos don't parasitize them. Davies and Brooke's interpretation would presumably be that reed buntings have come out the other side of a long ancestral arms race with cuckoos, which they eventually won. Dunnocks are near the beginning of
their arms race. Robins are slightly more advanced in theirs. Meadow pipits, reed warblers and pied wagtails are in the middle of theirs. When we say dunnocks have only just begun their arms race with cuckoos, 'only just' has to be interpreted with evolutionary timescales in mind. By human standards the association could still be quite old. The Oxford English Dictionary quotes a 1616 reference to the Heisugge (archaic word for hedge sparrow or dunnock) as 'a bird which hatcheth the Cuckooes egges'. Davies notes the following lines in King Lear I, iv, written a decade earlier:
For, you trow, nuncle,
The hedge-sparrow fed the cuckoo so long,
That its had it head bit off by it young.
And in the fourteenth century Chaucer wrote of the cuckoo's treatment of the dunnock in The Parliament of Fowls:
'Thou mortherere of the hey sage on the braunche That broughte the forth, thow rewthelees glotoun! '
Although dunnock, hedge sparrow and heysoge are all given as synonyms in the dictionary, I can't help wondering how far we should rely on medieval ornithology. Chaucer himself was usually a rather precise user of language, but nevertheless the name sparrow has at times been given to what today is technically called an LBB (little brown bird). This may have been Shakespeare's meaning in the following, from Henry IV Part I, V, i:
And, being fed by us, you used us so
As that ungentle gull the cuckoo's bird,
Useth the sparrow - did oppress our nest
Grew by our feeding to so great a bulk
That even our love durst not come near your sight For fear of swallowing;
Sparrow, on its own, would nowadays mean the house sparrow, Passer domesticus, which is never parasitized by cuckoos. Despite its alternative name hedge sparrow, the dunnock, Prunella modularis, is unrelated; it is a 'sparrow' only in the loose sense of being a little brown bird. But
anyway, even if we take Chaucer's evidence as showing that the arms race between cuckoos and dunnocks really does go back at least to the fourteenth century, Davies and Brooke cite theoretical calculations, taking into account the comparative rarity of cuckoos, suggesting that this is still sufficiently recent in evolutionary terms to account for the apparent naivety of dunnocks when faced with cuckoos.
Before we leave cuckoos, here's an interesting thought. There could be, simultaneously existing, more than one gens of, say, robin cuckoos, who have built up their egg mimicry. ' independently. Since there is no gene flow between them as far as Y chromosomes are concerned, there could be accurate egg mimics coexisting with less accurate egg mimics. All are capable of mating with the same males but they don't share the same Y chromosomes. The accurate mimics would be descended from a female who moved into parasitizing robins a long time ago. The less accurate ones would be descended from a different female who moved into robins, possibly from a different predecessor host species, more recently.
Ants, termites and other social insect species are odd in a different way. They have sterile workers, often divided into several 'castes' - soldiers, media (middle-sized) workers, minor (small) workers, and so on. Every worker, whatever its caste, contains the genes that could have turned it into any other caste. Different sets of genes are switched on under different rearing conditions. It is by regulating these rearing conditions that the colony engineers a useful balance of different castes. Often the differences among castes are dramatic.
In the Asian ant species Pheidologeton diversus, the large worker caste (specialized for bulldozing smooth paths for other colony members) is 500 times heavier than the small caste, who do all the normal duties of a worker ant. The same set of genes equips a larva to grow up into either a Brobdingnagian or a Lilliputian, depending upon which ones are switched on. Honeypot ants are immobile storage vats, abdomens pumped up with nectar to transparent yellow spheres, hanging from the ceiling of the nest. The normal duties of an ants' nest, defence, foraging and, in this case, filling up the living vats, are done by normal workers whose abdomens are not swollen. The normal workers have genes that equip them to be honeypots, and honeypots, as far as their genes are concerned, could equally well be normal workers. As in the case of male and female, the visible differences in bodily form depend upon which genes are switched on. In this case it is determined by environmental factors, perhaps diet. Once again, the zoologist of the future could read out from the genes, but not the body, of any one member of the species a complete picture of the disparate lives of the different castes.
The European snail Cepaea nemoralis comes in a number of colours and patterns. The background shell colour can be any of six distinct shades (in order of dominance, in the technical genetic sense): brown, dark pink, light pink, very pale pink, dark yellow, light yellow. Overlaying this, there may be any number of stripes from zero to five. Unlike the case of the social insects, it is not true that every individual snail is genetically equipped to assume any of the different forms. Nor are these differences among snails determined by different environments of upbringing. Striped snails have genes that determine their number of stripes, dark pink individuals have genes that make them dark pink. But all the kinds can mate with each other.
The reasons for the persistence of many different types of snail (polymorphism), as well as the detailed genetics of the polymorphism itself, have been exhaustively studied by the English zoologists A. J. Cain and the late P. M. Sheppard with their school. A major part of the evolutionary explanation is that the species ranges over different habitats - woodland, grassland, bare soil - and you need a different colour pattern to be camouflaged against birds in each place. Beechwood snails contain an admixture of genes from grassland because they interbreed at the margins. A chalk downland snail has some genes that previously survived in the bodies of woodland ancestors; and their legacy, depending on the other genes in the snail, may be stripes. Our zoologist of the future would need to look at the gene pool of the species as a whole to reconstruct the full range of its ancestral worlds.
Just as Cepaea snails range over different habitats in space, so the ancestors of any species have changed their way of life from time to time. House mice, Mus musculus, today live almost exclusively in or around human habitations, as unwanted beneficiaries of human agriculture. But by evolutionary standards their way of life is recent. They must have fed on something else before there was human agriculture. Doubtless that something was sufficiently similar for their genetic skills to be pressed into service when the agricultural bonanza came along. Mice and rats have been described as animal weeds (incidentally, a good piece of poetic imagery, genuinely illuminating). They are generalists, opportunists, carrying genes that helped their ancestors to survive through probably a considerable range of ways of life; and pre-agricultural genes are in them yet. Anybody attempting to 'read' their genes may find a confusing palimpsest of ancestral world descriptions.
From earlier still, the DNA of all mammals must describe aspects of very ancient environments as well as more recent ones. The DNA of a camel was once in the sea, but it hasn't been there for a good 300 million years. It has spent most of recent geological history in deserts, programming bodies to withstand dust and conserve water. Like sandbluffs carved into
fantastic shapes by the desert winds, like rocks shaped by ocean waves, camel DNA has been sculpted by survival in ancient deserts, and even more ancient seas, to yield modern camels. Camel DNA speaks - if only we could understand the language - of the changing worlds of camel ancestors. If only we could read the language, the DNA of tuna and starfish would have 'sea' written into the text. The DNA of moles and earthworms would spell 'underground'. Of course all the DNA would spell many other things as well.
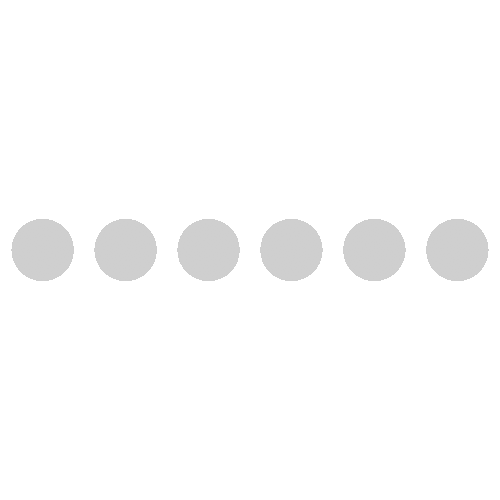